Superconducting Qubits A Quantum Leap
Superconducting qubits represent a leading contender in the race to build quantum computers. These tiny devices leverage the bizarre world of quantum mechanics, specifically the superconducting properties of certain materials at extremely low temperatures, to store and manipulate information in a fundamentally different way than classical bits. Their unique characteristics, from the intricate Josephson junctions at their heart to the sophisticated control techniques used to manage their quantum states, have propelled them to the forefront of quantum computing research, promising revolutionary advancements across diverse fields.
Understanding superconducting qubits involves delving into the physics of superconductivity, exploring different qubit designs like transmons and flux qubits, and grasping the methods used to control and measure their delicate quantum states. The challenges of maintaining coherence, scaling up to large numbers of qubits, and overcoming technological hurdles are significant but not insurmountable, as ongoing research continues to refine techniques and materials.
The potential applications, ranging from drug discovery and materials science to breaking current encryption standards, are immense, making the study of superconducting qubits a fascinating and vital area of scientific inquiry.
Introduction to Superconducting Qubits
Superconducting qubits are artificial atoms built using superconducting circuits, leveraging the principles of quantum mechanics to perform computations. Unlike classical bits representing 0 or 1, qubits can exist in a superposition, representing both 0 and 1 simultaneously. This allows quantum computers to explore vastly more possibilities than classical computers, potentially solving currently intractable problems in fields like materials science, drug discovery, and cryptography.
Their operation relies on manipulating the quantum states of these circuits using precisely controlled microwave pulses.The behavior of superconducting qubits stems from the unique properties of superconductors at extremely low temperatures. Below a critical temperature, certain materials exhibit zero electrical resistance and perfect diamagnetism (the Meissner effect). This allows for the creation of persistent currents and highly coherent quantum states necessary for qubit operation.
These states are fragile and require careful shielding from external noise to maintain their coherence.
Types of Superconducting Qubits
Several types of superconducting qubits have been developed, each with its strengths and weaknesses. The choice of qubit architecture often depends on the specific application and the trade-off between coherence time, controllability, and scalability.
- Transmon Qubits: These are currently the most widely used type of superconducting qubit. They are based on a Cooper-pair box (CPB) design but are engineered to have a larger capacitance, reducing sensitivity to charge noise. This results in longer coherence times, making them suitable for more complex quantum algorithms.
- Flux Qubits: These qubits utilize the magnetic flux threading a superconducting loop as their degree of freedom. Their energy levels are controlled by changing the external magnetic flux. Flux qubits are less susceptible to charge noise than CPB-based qubits but are more sensitive to magnetic flux noise.
- Phase Qubits: These qubits leverage the phase difference across a Josephson junction as their quantum bit. They are characterized by a relatively simple design but can be more sensitive to noise compared to transmons.
Historical Development of Superconducting Qubit Technology
The development of superconducting qubits has been a gradual process spanning several decades. Early experiments with Josephson junctions laid the groundwork for the creation of artificial atoms. Significant milestones include:
- Early 1980s – 1990s: Initial theoretical proposals and experimental demonstrations of superconducting qubits based on Josephson junctions. These early devices had short coherence times, limiting their potential.
- Early 2000s: The development of the Cooper-pair box (CPB) and the subsequent improvement of coherence times marked a significant advancement. This led to the exploration of more complex quantum algorithms.
- Mid-2000s – Present: The emergence of the transmon qubit, with its improved coherence properties, significantly boosted the field. Significant progress has been made in increasing qubit coherence times, developing more sophisticated control techniques, and scaling up the number of qubits in a quantum processor. Companies and research institutions are actively working towards building larger and more powerful superconducting quantum computers.
Physical Characteristics and Design
Superconducting qubits, the fundamental building blocks of quantum computers, are remarkably small devices relying on the bizarre properties of superconductivity at extremely low temperatures. Their design carefully balances the need for strong quantum coherence with the ability to control and manipulate their quantum states. This involves a precise selection of materials and a sophisticated arrangement of components.Superconducting qubits leverage the unique behavior of materials exhibiting zero electrical resistance and perfect diamagnetism below a critical temperature.
This allows for the creation of persistent currents and highly sensitive quantum circuits. The physical structure of these qubits is typically fabricated on a silicon or sapphire substrate using techniques like photolithography and electron-beam lithography. These processes create microscopic circuits with features only a few micrometers in size. Common superconducting materials include aluminum (Al), niobium (Nb), and sometimes, more exotic materials depending on the specific qubit design.
Josephson Junctions in Superconducting Qubits
The heart of a superconducting qubit is the Josephson junction. This is a weak link between two superconducting electrodes, typically formed by a thin insulating barrier (like aluminum oxide) sandwiched between two superconducting layers. The crucial role of the Josephson junction is to allow the passage of a superconducting current via the quantum mechanical tunneling effect. This tunneling effect is described by the Josephson relations, which govern the relationship between the current and the phase difference across the junction.
This phase difference acts as the qubit’s quantum degree of freedom, allowing it to exist in a superposition of states. The precise control of the current through the junction allows for manipulation of the qubit’s state. The junction’s properties, including its critical current and capacitance, are crucial parameters determining the qubit’s energy levels and coherence times.
Schematic Diagram of a Transmon Qubit
The following table illustrates a simplified schematic of a common type of superconducting qubit, the transmon. This is a widely used design due to its relatively long coherence times.
Component | Description |
---|---|
Superconducting Islands | Two larger superconducting islands (typically Aluminum) forming the main superconducting elements. These islands are capacitively coupled. |
Josephson Junction | A weak link (a thin insulating layer of Aluminum oxide) connecting the two superconducting islands. This allows for superconducting current to tunnel between the islands, and is the source of the qubit’s anharmonicity. |
Capacitors | Capacitors (often formed by overlapping metallic layers) are used to control the energy levels of the qubit. They provide additional capacitance to the junction, influencing the qubit’s frequency. |
Control Lines | These lines (often made of superconducting materials) allow for external control of the qubit’s state via microwave pulses. One line is typically used for driving the qubit transitions, while others might be used for flux control. |
Ground Plane | A large, grounded superconducting layer provides a stable reference for the qubit circuit. |
Qubit Control and Measurement
Controlling and measuring the quantum state of a superconducting qubit is crucial for building quantum computers. These processes involve manipulating the qubit’s energy levels and then extracting information about its state. Precise control and accurate measurement are essential for reliable quantum computation.Qubit control relies on manipulating the qubit’s energy levels using microwave pulses. These pulses, precisely tailored in frequency, amplitude, and duration, induce transitions between the qubit’s energy states, effectively changing the quantum state.
Measurement, on the other hand, involves extracting information about the qubit’s state without destroying the superposition. This is achieved through various techniques that indirectly reveal the qubit’s energy level.
Microwave Pulse Control
Microwave pulses are the primary method for controlling superconducting qubits. These pulses are applied to the qubit through a transmission line, exciting transitions between the ground and excited states. The frequency of the microwave pulse is carefully tuned to match the energy difference between the two states, ensuring efficient transitions. The pulse duration and amplitude determine the probability of transitioning to the excited state.
By precisely controlling these parameters, we can create specific quantum superpositions of the ground and excited states. For example, a short, low-amplitude pulse might create a superposition with a high probability of remaining in the ground state, while a longer, high-amplitude pulse would favor the excited state.
Qubit Measurement Techniques
Measuring the state of a superconducting qubit typically involves coupling it to a resonator. This resonator is a circuit element that interacts with the qubit, changing its properties based on the qubit’s state. The most common method uses a microwave resonator. When the qubit is in its excited state, it affects the resonator’s transmission characteristics, causing a measurable change in the signal passing through it.
By measuring the change in the resonator’s transmission, we indirectly determine the qubit’s state. This is often referred to as dispersive readout. Another technique involves directly measuring the qubit’s energy level through a process called direct current (DC) readout, which detects the change in the qubit’s current.
Comparison of Control and Measurement Techniques
Technique | Control | Measurement | Advantages | Disadvantages |
---|---|---|---|---|
Microwave Pulse Control | Applies microwave pulses to induce transitions between energy levels. | Not directly involved in measurement; often used in conjunction with dispersive readout. | High precision, versatile, allows for creation of complex superpositions. | Requires careful calibration and control of pulse parameters. |
Dispersive Readout | Indirectly influences the measurement process by altering the resonator. | Measures changes in resonator transmission properties to infer qubit state. | Relatively fast and efficient, good fidelity. | Susceptible to noise and crosstalk from neighboring qubits. |
DC Readout | Indirectly influences the measurement process by changing the qubit’s current. | Measures the change in the qubit’s DC current. | Simple setup, direct measurement of the qubit’s state. | Lower speed and fidelity compared to dispersive readout. |
Coherence and Decoherence: Superconducting Qubits
Superconducting qubits, while incredibly promising for quantum computing, are susceptible to noise and disturbances that limit their ability to maintain quantum information. Understanding coherence and decoherence is crucial for building practical quantum computers. Coherence refers to the qubit’s ability to maintain its quantum state (superposition or entanglement) over time, while decoherence is the process by which this quantum information is lost due to interactions with the environment.The coherence of a superconducting qubit is determined by several key factors.
The quality of the fabrication process plays a significant role, as imperfections in the qubit’s structure can introduce energy levels that lead to relaxation and dephasing. The qubit’s environment, including the substrate on which it’s fabricated and the surrounding electromagnetic fields, also contributes significantly to its coherence. Minimizing these environmental interactions is vital for extending coherence times.
The qubit’s design itself, particularly its energy level spacing and susceptibility to noise, influences its coherence properties.
Factors Affecting Superconducting Qubit Coherence
Several factors contribute to the impressive coherence times observed in state-of-the-art superconducting qubits. High-quality materials and precise fabrication techniques minimize defects that could act as scattering centers for quasiparticles. Sophisticated shielding and filtering strategies suppress external electromagnetic noise. Furthermore, advanced qubit designs, such as transmon qubits, are inherently less susceptible to charge noise compared to earlier designs like Cooper-pair boxes.
Sources of Decoherence in Superconducting Qubits
Decoherence in superconducting qubits stems from several sources. One major contributor is charge noise, which arises from fluctuating electric fields in the environment. These fluctuations cause the qubit’s energy levels to shift, leading to dephasing. Flux noise, arising from fluctuating magnetic fields, similarly affects the qubit’s energy levels and contributes to decoherence. Phonon interactions, involving the coupling of the qubit to vibrational modes in the substrate, can also lead to energy relaxation.
Finally, quasiparticle tunneling, the movement of individual electrons across the superconducting junctions, can cause transitions between the qubit’s energy levels. These decoherence mechanisms often act in combination, making it challenging to isolate their individual contributions.
Strategies for Improving Qubit Coherence Times
Improving coherence times is paramount for advancing superconducting qubit technology. A number of strategies are employed to extend coherence:
- Material Purity and Fabrication Techniques: Using high-purity materials and advanced fabrication techniques like electron-beam lithography minimizes structural defects that act as sources of decoherence.
- Improved Shielding and Filtering: Implementing effective shielding against external electromagnetic noise and filtering of unwanted frequencies reduces the impact of charge and flux noise.
- Qubit Design Optimization: Designing qubits with reduced sensitivity to charge and flux noise, such as transmon qubits, significantly enhances their coherence properties. This often involves engineering specific energy level spacing and reducing the qubit’s susceptibility to environmental fluctuations.
- Environmental Engineering: Careful design of the qubit’s environment, including the choice of substrate and the use of dielectric materials with low loss tangents, can suppress phonon-mediated decoherence.
- Dynamic Error Correction: Implementing quantum error correction codes can mitigate the effects of decoherence by encoding quantum information redundantly and actively correcting errors as they occur. This is a more complex approach but offers significant potential for improving the fidelity of quantum computations.
Quantum Gates and Algorithms
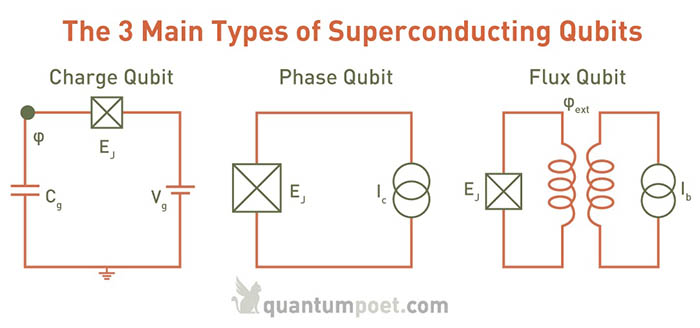
Source: quantumpoet.com
Superconducting qubits, with their remarkable coherence properties, form the foundation for implementing quantum gates, the fundamental building blocks of quantum computation. These gates manipulate the qubit’s quantum state, allowing for the execution of complex quantum algorithms that offer potential advantages over classical computing for specific tasks.Quantum gates are implemented on superconducting qubits by carefully controlling the qubit’s parameters. This control is typically achieved through microwave pulses applied to the qubit’s control lines.
Superconducting qubits are a key component in building powerful quantum computers. These computers will revolutionize many fields, and their impact on cybersecurity is a major concern, as discussed in this article about Quantum AI’s impact on cybersecurity and data encryption. Understanding this impact is crucial for developing robust defenses against future quantum-based attacks, and further research into superconducting qubits is essential to both advance and protect quantum computing.
By precisely shaping the amplitude, frequency, and duration of these pulses, we can induce specific unitary transformations on the qubit, corresponding to the desired quantum gate. For example, a single-qubit rotation gate might involve a microwave pulse that changes the phase or amplitude of the qubit’s superposition state. Two-qubit gates, which entangle multiple qubits, are typically implemented through controlled interactions mediated by a coupler element connecting the qubits.
Superconducting qubits, with their potential for incredibly fast and powerful quantum computing, are attracting significant attention. Their development is intertwined with advancements in other high-tech fields, like the 6G research and development which will need incredibly efficient data processing. Ultimately, the breakthroughs in both areas could revolutionize how we handle and transmit information, further accelerating the progress of superconducting qubit technology itself.
These interactions can be engineered to generate specific entanglement patterns, allowing for the creation of multi-qubit entangled states.
Single-Qubit and Two-Qubit Gate Implementations
Single-qubit gates, such as the Hadamard (H) gate, the Pauli-X (NOT) gate, and rotations around the Bloch sphere axes (Rx, Ry, Rz), are fundamental operations that modify the state of a single qubit. These gates are implemented by applying precisely calibrated microwave pulses to the qubit’s control lines. The specific pulse parameters (amplitude, frequency, duration, and phase) determine the specific unitary transformation performed on the qubit.
Two-qubit gates, such as the Controlled-NOT (CNOT) gate, are essential for creating entanglement between qubits. These gates are implemented through controlled interactions between qubits, often mediated by a coupler element that allows for the controlled exchange of energy or phase between the qubits. The strength and duration of the interaction determine the probability of successful entanglement.
Quantum Algorithms on Superconducting Qubits
Several quantum algorithms have been successfully executed on superconducting qubit processors. These include Shor’s algorithm for factoring large numbers (although a full-scale implementation for realistically large numbers is still beyond current capabilities), Grover’s algorithm for searching unsorted databases, and quantum simulations of physical systems. Quantum simulations are particularly promising, as they allow researchers to model complex systems that are intractable for classical computers, such as the behavior of molecules or materials with intricate interactions.
Experiments demonstrating these algorithms often involve a small number of qubits, but they represent significant milestones towards building larger, more powerful quantum computers.
Example Quantum Circuit: Quantum Teleportation
The following table illustrates a simple quantum circuit for quantum teleportation using two entangled qubits and a classical communication channel. This protocol allows the transfer of the quantum state of one qubit to another qubit, even if they are spatially separated.
Qubit 1 (Alice’s qubit) | Qubit 2 (Entangled qubit) | Qubit 3 (Bob’s qubit) | Measurement |
---|---|---|---|
H | |||
CNOT | |||
Measurement | Measurement | Classical Communication | |
Corrections based on measurements |
Scalability and Challenges
Building a large-scale, fault-tolerant quantum computer using superconducting qubits presents significant technological hurdles. While individual qubits have demonstrated impressive performance, scaling up to thousands or millions of qubits while maintaining their coherence and control presents a formidable challenge. The complexity increases exponentially, demanding innovative solutions in fabrication, control electronics, and error correction.The primary challenge lies in maintaining the delicate quantum states of individual qubits within a large interconnected system.
As the number of qubits increases, the complexity of the control electronics, wiring, and cryogenic infrastructure escalates dramatically, leading to increased cost and susceptibility to errors. Furthermore, the inherent fragility of superconducting qubits to noise and environmental factors becomes more pronounced in larger systems.
Qubit Connectivity and Control
Connecting a large number of qubits in a scalable and efficient manner is crucial. Current approaches often involve complex wiring schemes, which can lead to crosstalk between qubits and increased susceptibility to noise. The need for individual control lines for each qubit further exacerbates the complexity of the control electronics. For example, a system with 1000 qubits would require thousands of control lines, demanding sophisticated and highly integrated control systems.
This complexity also increases the challenges in calibration and tuning the system.
Material Science and Fabrication
The fabrication of superconducting qubits requires extremely precise and high-yield manufacturing processes. Maintaining uniformity and consistency across a large number of qubits is essential for ensuring their performance and coherence. Imperfections in the fabrication process can lead to variations in qubit properties, resulting in reduced performance and increased error rates. Moreover, the integration of qubits with control electronics and other components requires advanced microfabrication techniques.
The development of new materials with improved superconducting properties and reduced susceptibility to noise is crucial for enhancing the performance and scalability of superconducting qubits.
Error Correction and Fault Tolerance
Superconducting qubits are susceptible to various sources of noise and decoherence, leading to errors in computation. Implementing effective error correction codes is essential for building fault-tolerant quantum computers. However, implementing such codes requires a significant overhead in terms of the number of qubits and control complexity. For instance, surface codes, a promising approach to fault-tolerant quantum computation, require a substantial number of physical qubits to encode a single logical qubit.
This necessitates even larger-scale systems to perform meaningful computations.
Potential Solutions to Scalability Challenges
Overcoming these challenges requires a multi-pronged approach. Several potential solutions are being actively explored:
- Improved qubit design: Developing qubits with enhanced coherence times and reduced sensitivity to noise. This could involve exploring new materials, improved fabrication techniques, or novel qubit architectures.
- Integrated control electronics: Developing highly integrated and miniaturized control electronics to reduce wiring complexity and crosstalk. This could involve using advanced chip-packaging techniques or developing new control schemes.
- Advanced cryogenic systems: Designing more efficient and scalable cryogenic systems to cool and maintain the large number of qubits at their operating temperatures. This could involve exploring new refrigeration technologies or improving the thermal management of the system.
- New qubit architectures: Exploring alternative qubit architectures that are inherently more scalable, such as those based on 2D or 3D arrays, allowing for more efficient connectivity and control.
- Efficient error correction codes: Developing more efficient error correction codes that require fewer physical qubits to encode a logical qubit. This requires advancements in both theoretical and experimental aspects of quantum error correction.
Applications of Superconducting Qubits
Superconducting qubits, with their relatively long coherence times and established fabrication techniques, are poised to revolutionize several fields. Their unique properties make them particularly well-suited for tackling complex computational problems beyond the reach of classical computers. This section explores their potential impact across diverse applications.Superconducting qubits offer a compelling pathway towards building powerful quantum computers capable of solving currently intractable problems.
Their potential applications span various sectors, from materials science and drug discovery to cryptography and financial modeling. These applications leverage the qubit’s ability to perform quantum algorithms, offering speedups over classical methods for specific tasks.
Quantum Computing Applications
The most direct application of superconducting qubits lies in building large-scale, fault-tolerant quantum computers. These computers will be capable of solving problems currently intractable for even the most powerful supercomputers. Specific examples include factoring large numbers (relevant to cryptography), simulating quantum systems (crucial for materials science and drug discovery), and optimizing complex logistics problems (useful in supply chain management and transportation).
The potential impact on various industries is vast and still largely unexplored. For instance, the development of new drugs and materials could be significantly accelerated through quantum simulations, potentially leading to breakthroughs in medicine and technology. Similarly, improvements in logistics optimization could lead to significant economic gains and societal benefits.
Quantum Simulation Applications
Quantum simulation is another crucial area where superconducting qubits excel. Their ability to precisely control and manipulate individual qubits allows researchers to simulate the behavior of complex quantum systems, such as molecules or materials, that are impossible to study using classical methods. This capability is invaluable in various fields. For example, simulating the behavior of molecules can lead to the design of new catalysts, more efficient solar cells, and novel drugs.
Simulating the properties of materials could lead to the discovery of new superconductors or other advanced materials with unique functionalities. The development of highly accurate and efficient quantum simulators promises breakthroughs in fundamental scientific understanding and technological innovation.
Current Research and Development Efforts
Significant research and development efforts are underway globally to explore and expand the applications of superconducting qubits. These efforts are focused on both improving the performance of individual qubits and developing larger-scale quantum processors.
- Google’s Quantum AI team is actively developing superconducting qubit-based quantum computers, aiming to achieve quantum supremacy and explore applications in various fields, including materials science and optimization problems.
- IBM’s Quantum Computing group is building and providing access to a series of superconducting quantum processors through the cloud, enabling researchers worldwide to explore different quantum algorithms and applications.
- Rigetti Computing is focusing on developing modular quantum computers using superconducting qubits, aiming to scale up the system size and improve the performance of quantum algorithms.
- Numerous academic institutions and national laboratories are conducting research on improving qubit coherence times, developing new quantum gates, and exploring novel quantum algorithms suitable for superconducting qubit architectures.
Comparison with Other Qubit Technologies
Superconducting qubits, while showing immense promise in quantum computing, are not the only game in town. Several other qubit technologies are being actively pursued, each with its own strengths and weaknesses. Comparing these different approaches is crucial for understanding the overall landscape of quantum computing development and identifying the most promising pathways towards building a fault-tolerant quantum computer.Superconducting qubits, trapped ions, and photonic qubits represent three of the most advanced qubit platforms.
Superconducting qubits are a key component in building powerful quantum computers. Their development is directly tied to the future of quantum AI, and understanding this connection is crucial. To fully grasp the implications, check out this article on The impact of quantum AI on the job market and workforce development to see how these advancements will reshape industries.
Ultimately, the progress made with superconducting qubits will influence the scale and speed of this transformation.
Each leverages different physical principles to encode and manipulate quantum information, leading to distinct advantages and challenges in terms of scalability, coherence times, and control fidelity.
Superconducting Qubits Compared to Trapped Ions and Photonic Qubits
Trapped ion qubits utilize individual ions confined in electromagnetic traps, with their internal energy levels representing the qubit states. Photonic qubits, on the other hand, encode information in the polarization or other properties of photons. Superconducting qubits, as discussed previously, leverage the quantum properties of superconducting circuits.
Characteristic | Superconducting Qubits | Trapped Ions | Photonic Qubits |
---|---|---|---|
Qubit Coherence Time | Microseconds to milliseconds | Milliseconds to seconds | Nanoseconds to microseconds |
Scalability | Potentially high, but faces challenges in wiring and control | Moderate, limited by the number of ions that can be effectively trapped and controlled | High potential, but challenges in creating complex entangled states and efficient interconnects |
Control Fidelity | High, approaching 99.9% for single-qubit gates | Very high, exceeding 99.9% for single-qubit gates | Moderate to high, depending on the specific implementation |
Qubit Fabrication | Uses advanced lithographic techniques, similar to semiconductor fabrication | Requires specialized ion traps and laser systems | Relies on advanced optical components and sources |
Cost and Complexity | Relatively high fabrication cost, but control systems can be relatively simpler | High cost and complexity due to the specialized equipment required | Moderate to high cost, depending on the specific implementation |
Advantages and Disadvantages of Superconducting Qubits
Superconducting qubits offer several advantages, including their relatively long coherence times (compared to photonic qubits), potential for high scalability through integration with existing semiconductor fabrication techniques, and the relatively high fidelity of control operations. However, they also face challenges, including the need for cryogenic cooling to maintain superconductivity, the complexity of controlling many qubits simultaneously, and susceptibility to noise from the environment.
In contrast, trapped ion systems boast exceptional coherence times and high control fidelity but face scalability limitations. Photonic systems offer inherent advantages in terms of scalability and long-distance communication, but currently have shorter coherence times and lower control fidelity than superconducting or trapped ion systems. The “best” technology is ultimately application-dependent and subject to ongoing research and development.
Future Directions and Outlook
Superconducting qubits, while already demonstrating impressive capabilities, are poised for significant advancements in the coming years. Further miniaturization, improved coherence times, and the development of more robust fabrication techniques will unlock their full potential across diverse scientific and technological domains. The convergence of materials science, quantum engineering, and computational algorithm development promises a transformative impact on fields ranging from medicine and materials discovery to artificial intelligence and cryptography.The future of superconducting qubit technology hinges on several key areas of development.
These advancements will not only enhance the performance of individual qubits but also pave the way for larger-scale, fault-tolerant quantum computers.
Improved Qubit Coherence and Control
Longer coherence times are crucial for performing complex quantum computations. Current research focuses on improving materials and fabrication techniques to minimize decoherence sources, such as environmental noise and material imperfections. For instance, advancements in fabrication processes, including the use of higher-quality superconducting materials and improved device designs, are expected to lead to coherence times exceeding milliseconds, significantly enhancing the computational power of superconducting qubits.
This would enable the execution of more complex quantum algorithms and simulations, pushing the boundaries of what’s computationally feasible. For example, the development of novel materials with reduced dielectric loss and improved surface quality would dramatically enhance coherence times.
Scalability and Integration
Building large-scale quantum computers requires efficient integration of many qubits while maintaining their individual control and connectivity. Current efforts focus on developing scalable architectures, such as 2D and 3D arrays, and improving the fidelity of qubit control and readout. One promising approach involves the development of integrated circuits with on-chip microwave control and readout components, minimizing signal loss and simplifying the overall system.
Superconducting qubits, with their exceptional coherence times, are key components in building powerful quantum computers. These advancements are directly fueling progress in areas like drug discovery, as highlighted by the exciting work detailed in this article on Quantum AI applications in drug discovery and development. Ultimately, improved qubit technology will lead to even more breakthroughs in simulating molecular interactions and accelerating the development of new medicines.
This will significantly reduce the complexity and cost associated with controlling and monitoring a large number of qubits, making large-scale quantum computation more practical. The development of high-density qubit arrays, potentially exceeding millions of qubits, is a key target for this area.
Advancements in Materials Science and Engineering
The performance of superconducting qubits is intrinsically linked to the properties of the superconducting materials used in their fabrication. Research into new superconducting materials with higher critical temperatures and improved properties, such as reduced surface loss and enhanced resilience to environmental noise, is crucial for improving qubit performance. For example, the exploration of novel materials like topological superconductors holds the promise of inherently more robust and fault-tolerant qubits.
Furthermore, advancements in nanofabrication techniques are needed to create qubits with precise geometries and improved surface quality, minimizing defects and enhancing coherence. The use of advanced lithographic techniques and material deposition methods will play a critical role in achieving this goal. Improved understanding of material interfaces and the development of surface passivation techniques will also help minimize noise and improve coherence.
Impact on Various Fields
The development of scalable and fault-tolerant superconducting quantum computers will have a profound impact on various fields. In drug discovery and materials science, quantum simulations will enable the design of new drugs and materials with unprecedented precision. In finance, quantum algorithms can revolutionize portfolio optimization and risk management. In artificial intelligence, quantum machine learning algorithms can accelerate the development of more powerful and efficient AI systems.
Furthermore, quantum cryptography based on superconducting qubits promises enhanced security for communication networks. For example, quantum simulations could accelerate the discovery of new catalysts for sustainable energy technologies or the development of novel high-temperature superconductors for various applications.
Last Word
In conclusion, superconducting qubits offer a compelling pathway towards realizing the transformative potential of quantum computing. While challenges remain in scaling up and improving coherence times, the ongoing progress in materials science, control techniques, and error correction strategies is incredibly promising. The unique properties of these devices, combined with the intense research efforts focused on their development, strongly suggest that superconducting qubits will play a crucial role in shaping the future of computation and numerous other scientific and technological endeavors.
The journey towards fault-tolerant quantum computers is underway, and superconducting qubits are at the forefront of this exciting revolution.
Expert Answers
What is the temperature at which superconducting qubits operate?
Superconducting qubits operate at extremely low temperatures, typically near absolute zero (around -273°C or 0 Kelvin), to maintain their superconducting state.
How are errors corrected in superconducting qubits?
Error correction in superconducting qubits is an active area of research. Techniques involve encoding quantum information redundantly across multiple qubits to detect and correct errors caused by decoherence.
What are the main limitations of superconducting qubits?
Major limitations include susceptibility to noise, challenges in scaling up to large numbers of qubits, and the need for complex and expensive cryogenic infrastructure.
What is the role of a Josephson junction?
A Josephson junction is a crucial component, acting as a weak link in the superconducting circuit, enabling control over the qubit’s quantum state through manipulation of the current flowing through it.
Are superconducting qubits already being used commercially?
Not yet for general-purpose quantum computing. Current applications are primarily research-focused, with some early explorations in specific niche areas.